The stars still have secrets. we know why they shine, and we know why they twinkle, but we still do not know why they move the way they move. The problem has been with us for the better part of a century. In the 1930s Swiss astronomer Fritz Zwicky observed that some galaxies in a cluster of about 1,000 fly surprisingly fast around their common center of mass. Even with generous estimates of the individual galaxies’ masses, they did not add up enough to account for this motion. Zwicky fixed the mismatch by conjecturing the existence of a new kind of matter: “dark matter.”
In the 1970s American astronomer Vera Rubin, who died in 2016, saw the same thing happening in single galaxies. The velocities of stars far out from the center of a galaxy remained roughly the same as those closer in, when astronomers would have expected them to slow down because of the dwindling gravity at the galaxy’s far reaches. Again, the visible mass alone was not sufficient to explain the observations. Rubin concluded that in galaxies, too, dark matter must be present.
Since then, even more evidence has accumulated that we must be missing something. The tiny temperature fluctuations in the cosmic background radiation astronomers see pervading space, as well as the gravitational bending of light around galaxies and galaxy clusters and the formation of the cosmic web of large-scale structure throughout space, confirm that normal matter alone cannot explain what we see.
For many decades the most popular hypothesis has been that dark matter is composed of new, so far undetected particles that do not interact with light. The alternative explanation that we have the right particles but the wrong laws of gravity has received little attention.
Thirty years ago this stance was justified. The idea of particle dark matter gained traction because back then physicists had other reasons to believe in the existence of new particles. Around the 1950s and 1960s physicists realized that the protons, neutrons and electrons that make up atoms are not the only particles out there. Over the next decades particle accelerators started turning up new particles left and right; these came to make up the Standard Model of particle physics and opened theorists’ minds to even more possibilities. For instance, efforts to unify the fundamental forces of nature into a single force required theorizing a set of new particles, and the concept of supersymmetry, developed in the 1970s, predicted a mirror particle for every known particle in the universe. Some of these theorized particles would make good dark matter candidates. Another suspect for the role was a particle called the axion, invented to explain the smallness of a parameter in the Standard Model. But after three decades of failed attempts to detect any of these particles, ignoring alternative hypotheses is no longer reasonable.
Meanwhile the idea that dark matter is made of particles has come under pressure from an entirely different direction. New astrophysical data gathered and analyzed by one of us (McGaugh), as well as others, conflict with particle dark matter predictions. It is also becoming increasingly clear that some old problems with the dark matter paradigm persist even after many attempts to resolve them.
Updating the equations of gravity is still a viable way forward. Rather than adding particles to the universe to account for the extra gravity that seems to exist in galaxies and clusters, we can instead stick with the known particles but increase the force they exert on one another. Often dismissed and overlooked, modified gravity, as these theories are called, has never been ruled out. Now is a good opportunity to reconsider the option that we have been looking for the wrong thing in the wrong places. It is time to have a closer look at modified gravity.
Tweaking gravity
First put forward by Israeli physicist Mordehai Milgrom in 1983, modified gravity changes the mathematical rules that govern how the force of gravity arises from mass. In most cases (that is, in nonextreme situations where Newtonian gravity is a good approximation), we describe this force by the inverse square law: the strength of gravity between two objects depends on their masses and decreases with the inverse square of the distance between them. This law is a classic and shows up all over physics, from equations describing how light intensity drops off with distance to rules describing sound pressure. But what if gravity does not always follow the inverse square law? What if the equations, in certain circumstances, should be tweaked?
Milgrom’s first proposal—modified Newtonian dynamics (MOND)—dealt only with the Newtonian laws of gravity. But Einstein’s general theory of relativity taught us that gravity is not a force and is instead caused by the curvature of space and time. This limitation of the original MOND was likely a key reason many physicists did not take the idea seriously. But we now know several ways to make MOND compatible with general relativity, each using different types of fields that behave slightly differently to describe how gravitational attraction arises from mass. It is these 10 or so more complete theories that we collectively refer to as modified gravity. Dismissing them on purely theoretical grounds is no longer warranted. Another objection to modified gravity is that its mathematical expression appears inelegant from the perspective of particle physics. Not only does it look unfamiliar, it is also more difficult to deal with than particle dark matter, which employs techniques taught as part of the standard curriculum. Although these factors help to explain the idea’s unpopularity, they are not scientific grounds for discounting it.
Despite the potential of modified gravity, however, scientists have put almost all their energy on this front into searching for dark matter. Since the mid-1980s dozens of projects have sought the rare interactions predicted between dark matter particles and normal matter. Such experiments place large tanks of liquefied noble gases or carefully prepared solids, kept at extremely low temperatures, in well-shielded environments such as underground mines to avoid contamination from cosmic radiation. Sensitive detectors patiently wait for telltale signs of a dark matter particle bouncing off an atomic nucleus in the liquid or solid target.
The most recent round of dark matter searches just concluded. The very sensitive Large Underground Xenon (LUX) experiment in South Dakota and PandaX-II (for Particle and Astrophysical Xenon Detector) in Sichuan Province in China, like all other dark matter detection experiments before them, recently reported no evidence for particles that could make up dark matter. The first results from XENON1T at Gran Sasso National Laboratory in Italy (an upgrade of XENON100, which was itself an upgrade of XENON10) were also negative. Neither has Super-Kamiokande in Japan seen any signal of protons decaying, which would be evidence for a unification of the fundamental forces and give credibility to the idea that unseen particles must exist. At the same time, scientists at the Large Hadron Collider (LHC) at CERN near Geneva have been looking for novel particles with the right properties for dark matter and have seen no signs of them. Besides the expected Higgs boson, the LHC has seen no new particles at all.
Of course, these negative results do not rule out dark matter. Theories for particle dark matter have become increasingly sophisticated, not to say contrived. To evade conflict with experimental null results, theorists now assume the particles interact with normal matter even less than originally thought. Some researchers have begun to conjecture new forces and additional particle species to go with the original new particles. This proliferation of unseen particles has become so common in the literature that they have been given a collective name: the “hidden sector.”
Comparing the theories
In the absence of any signs of new particles, we should ask how well the theories of dark matter and modified gravity, respectively, explain the evidence we do have from nature.
For the most part, the hypothesis that the universe contains about five times as much dark matter as normal matter works well to explain the cosmos around us. Although dark matter’s microscopic properties can be complicated, it follows simple equations in bulk. We can describe dark matter as behaving like a fluid without internal pressure, its one variable being the average density of particles in space.
Treating dark matter as a pressureless fluid suffices to reproduce the patterns we observe in the cosmic microwave background. It also does a good job with the formation of large-scale cosmic structures. As the early universe expanded and matter cooled, particle dark matter, because it cannot build up internal pressure, would have begun to clump under the pull of gravity faster than normal matter. Only later would the normal matter collect in the clouds of dark matter to form galaxies. This scenario fits well with some aspects of our observations.
Particle dark matter explains the motions of stars within galaxies when we distribute suitable amounts where needed; clusters of galaxies work out in much the same way. Because theorists can sprinkle dark matter so flexibly, they can make all current observations fit with the predictions of general relativity.
But this flexibility of particle dark matter is also its greatest shortcoming. Galaxies are not particles, and no two are alike. Each galaxy has its own history; each came about in its own delicate dance of billions of stars following the pull of gravitational attraction. Some young galaxies collide and form larger galaxies. Some do not. Some galaxies end up as spinning disks, some as elliptic puff balls. Sometimes dark matter catches a lot of normal matter in its gravitational pull; sometimes it does not. Because of these many variations, you would expect a ratio of dark matter to normal matter that differs from one galaxy to the next. You would expect variety, not strict rules. But the data beg to differ.
In 2016 McGaugh and his colleagues made thousands of measurements in more than 150 galaxies and compared the gravitational pull expected from the normal matter in them with the observed gravitational pull that presumably resulted from the dark matter and normal matter combined. What they found was surprising: a strong correlation between the two. In fact, a simple equation relates the apparent amount of dark matter to the amount of normal matter in each galaxy; deviations from the curve are small and few [see box below].
This correlation is difficult to reproduce with computer simulations that treat the two types of matter as independent components. Scientists can make the simulations fit the data, but they must insert many parameters that have to be carefully chosen. Modified gravity, in stark contrast, simply predicts this correlation. Because this scenario involves only one type of matter—normal matter—of course the total gravity closely follows the gravity caused by the visible matter. Milgrom even predicted this observation in the early 1980s.
Unusual galaxies
There are other problems with the dark matter hypothesis—for instance, “low-surface-brightness galaxies.” In these dim galaxies, visible matter is spread more thinly than in galaxies similar to the Milky Way.
The dark matter hypothesis originally led us to expect that galaxies with low surface brightness—that is, low amounts of visible matter—should also generally have low amounts of dark matter. Scientists assumed stars orbiting at large distances from the galactic center would move slower in these galaxies than in normal galaxies of the same size because there was less total gravity pulling the stars along their orbits. But when the data came in, this expectation turned out to be wrong. The outer stars in these unusual galaxies were moving just as fast as they do in normal ones, suggesting that there was actually quite a lot of matter in low-surface-brightness galaxies, despite the sparseness of the stars. It turns out that in these objects, the ratio of dark matter to normal matter must be much higher than originally expected. But why should that be?
Originally, the dark matter hypothesis offered no explanation. But as we noted already, it is a very flexible hypothesis, so when theorists sought ways to explain this odd situation, they found them.
To square the findings with the theory, scientists had to fine-tune the amount of dark matter in each galaxy to depend on the surface brightness of the stars: the dimmer the system, the more dark matter. Doing so required some mechanism to rid these galaxies of luminous matter while they formed, so that the matter ratio tilted in favor of dark matter. Currently the most popular method is to add “stellar feedback” to the computer simulations. Stellar feedback is caused by the pressure created when massive stars irradiate their surrounding gas with highly energetic photons, blow strong stellar winds and ultimately go supernova. These giant explosions can blow matter out of galaxies. And because dark matter interacts so weakly, this blowout would affect normal matter more than dark matter. Galaxies that happen to have many supernovae would thus end up with an increased ratio of dark matter.
But although we know stellar feedback plays an important role in the formation of stars and stellar clusters, its role during galaxy formation is less clear. To solve the problem with low-surface-brightness galaxies, supernovae’s energy must go almost entirely into pushing matter out of galaxies. Such a high level of efficiency, however, is strikingly implausible for a naturally occurring process. Modified gravity, on the other hand, predicts the observed outcome without involving feedback, just as it predicted the observed rotation speeds of stars in normal galaxies.
More problems
The issue with low-surface-brightness galaxies is far from the only shortcoming of particle dark matter. The theory predicts, for instance, a highly peaked density of matter in the cores of galaxies, in contrast with what we measure. It predicts many more small dwarf galaxies than we observe and fails to predict the way that galaxies and their satellite galaxies align along a single plane. These are just the most prominent disagreements. Modified gravity does better in all these areas.
The lack of density peaks in galactic cores, in particular, fits so badly with the dark matter story that when the data were new, many astrophysicists doubted they were correct. First, the theorists asserted that the resolution of the measurements was inadequate. When subsequent data settled the issue of resolution, they blamed other systematic errors. But after several more generations of observations obtained by multiple groups, the conclusion remains the same: dark matter does a bad job of explaining what we see at the centers of galaxies.
It is true that incorporating stellar feedback and other astrophysical effects into the computer simulations alleviates these issues. Because these extra processes add more parameters to the simulations, researchers can coax the software into producing galaxies that resemble what we observe reasonably well. These simulated galaxies can then also reproduce the observed correlation between the amount of particle dark matter and normal matter. What the computer simulations do not offer, however, is any explanation for the origin of this correlation.
And modified gravity has another advantage. In contrast to dark matter simulations, modified gravity can explain how small galaxies behave when trapped in the gravitational field of larger galaxies. For instance, its calculations have been enormously successful in predicting how a bunch of recently discovered dwarf galaxies swirl around our large neighbor galaxy, Andromeda. These tiny dwarfs are subject to a gravitational pull from their giant host that is stronger than their internal gravity. In such a situation, modified gravity offers a different prediction than it would if the dwarf galaxies were isolated, and it is this unique prediction that we find realized in the observations. Fitting this aspect of the data with particle dark matter, however, requires adding yet more assumptions to the computer simulations.
But let us be fair: Despite these many predictive successes, modified gravity has serious problems. Although it works across a huge range of different galaxy types, it cannot explain the motion of galaxy clusters very well. And on the behavior of the cosmos as a whole, modified gravity is mute. In these cases, particle dark matter works better. It accounts for the properties of the cosmic microwave background and the distribution of galaxies throughout the universe, where modified gravity has no answers. Yet discarding modified gravity because it does not address these situations misses the point. The theory has made successful predictions. Even if we do not understand why, ignoring it will not help.
Moving forward
At this point, both particle dark matter and modified gravity have advantages and shortcomings. Some recent theoretical developments suggest that maybe the truth is in between: a type of particle dark matter that can masquerade as modified gravity.
In 2015 Justin Khoury of the University of Pennsylvania and his colleagues found that some types of particle dark matter can become superfluids—fluids that flow with no resistance, in which quantum effects are dominant. When the superfluid dark matter collects in galaxies, its quantum properties can generate a long-range force that resembles modified gravity. The superfluid itself has a gravitational pull, but according to Khoury’s hypothesis, most of the effect we now assign to dark matter comes not from gravity but from the superfluid’s direct interaction with normal matter. This phenomenon would explain why the force we witness acting on normal matter in galaxies is hard for gravity to account for: it is not caused by gravity.
The idea that dark matter is a type of superfluid that mimics modified gravity also clarifies why modified gravity does not work well for galaxy clusters. Throughout most clusters, gravity is not strong enough to make the particles superfluid. In these situations, they behave like a normal fluid—that is, they behave like particle dark matter.
And as one of us (Hossenfelder) noticed by accident, the superfluid concept matches another line of research, pioneered by Erik Verlinde of the University of Amsterdam. Verlinde uses ideas from string theory to argue that the impression that the universe contains more matter than we can see is an illusion caused by the reaction of space to the presence of mass. Although this notion sounds entirely different from Khoury’s superfluid hypothesis, the key equation in both cases is almost the same.
This line of research is young and might turn out to be a dead end. But it exemplifies how having a closer look at modified gravity may help overcome the current phase of stagnation in the search for dark matter.
And new data should be available soon that will help determine the truth. Traditional particle dark matter, modified gravity and superfluid dark matter all make different predictions for low-surface-brightness galaxies that may become testable in the near future. The Dark Energy Survey currently identifies such galaxies, and the Large Synoptic Survey Telescope should find them by the hundreds when it comes online this year. The theories also differ when talking about the early universe, when the first galaxies were forming. These galaxies should be observable by the James Webb Space Telescope, which is set to launch in 2021, and future long-wavelength radio observations will probe the dark ages at still earlier epochs.
The advent of gravitational-wave astronomy is also giving us new clues. The Laser Interferometer Gravitational-wave Observatory (LIGO) recently detected gravitational waves caused by the collision of two neutron stars. At the same time, various telescopes observed light in different wavelengths emitted by the same event. These observations show, to excellent precision, that gravitational waves travel at the same speed as light. This finding has ruled out some, but certainly not all, variants of modified gravity.
Right now a few dozens of scientists are studying modified gravity, whereas several thousand are looking for particle dark matter. Perhaps modified gravity is wrong, but perhaps the scientific community is not putting in a good faith effort to know for sure. The universe has had a habit of surprising us; we should be prepared to greet what future data reveal with open minds. The stars may still have secrets, but they are under close surveillance.
A Collision Offers Clues
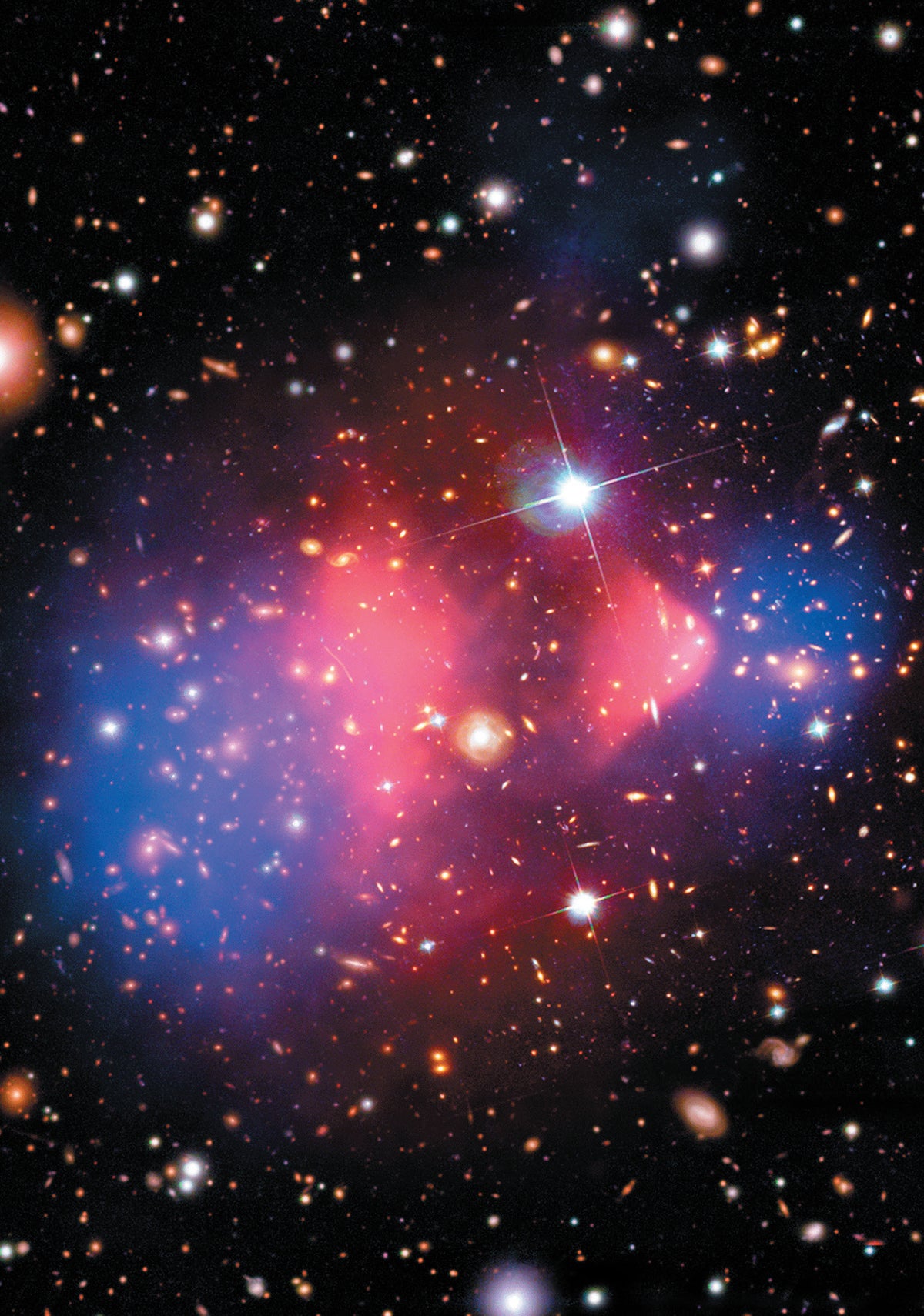
Credit: NASA, CXC, CfA and M. Markevitch et al. (x-ray); NASA, STScI, Magellan University of Arizona and D. Clowe et al. (optical); NASA, STScI, ESO WFI, Magellan, University of Arizona and D. Clowe et al. (lensing map)
The Bullet Cluster is a pair of galaxy clusters that crashed together long ago. It is a rare instance of a high-speed head-on collision. Images taken in visible and x-ray light (red), along with measurements of how gravity is bending light (gravitational lensing) (blue), reveal that in each cluster the center of the total mass and gravity is misplaced from the center of the visible mass.
Scientists often claim the Bullet Cluster is evidence for particle dark matter. Because such particles would interact less than normal matter, the collision would have allowed the clusters’ dark matter clouds to pass one another while the visible mass interacted with itself and lagged behind. This story matches what we observe, but it is crudely oversimplified.
In modified gravity, too, the point where gravitational attraction focuses can be displaced from the normal mass. This can occur because all forces, including gravity, are thought to be transmitted by a special type of particle. These particles have their own dynamical laws to fulfill. When modified gravity takes into account potential repercussions from these carrier particles, it can also predict what we see in the Bullet Cluster.
More important, this cluster is an extreme event and a statistical outlier. Its mere existence is difficult to explain both with particle dark matter and with modified gravity. Using it as evidence for or against either approach is an exercise in confirming our own biases.